In 1945, a young engineer named Wallace H. Coulter was particularly horrified by the devastation of the 1945 atomic bombings in Japan.1
Coulter had spent extensive time in hospitals, making him aware of how slow and laborious manual blood-cell counts were. This knowledge, combined with the reality that the radiation from the atomic bombs was going to dramatically increase blood diseases, led the young man to believe a blood cell counting device was needed to help track disease progression.
In 1948, Coulter unveiled his first prototype. Developed with the logic that if blood cells flow through a similiarly sized aperture, it would be possible to calculate the number and size of cells. This notion was based on the principles he had learned as an electrical engineering student, where particles in a solution will have a different electrical conductivity to that of the solution itself.
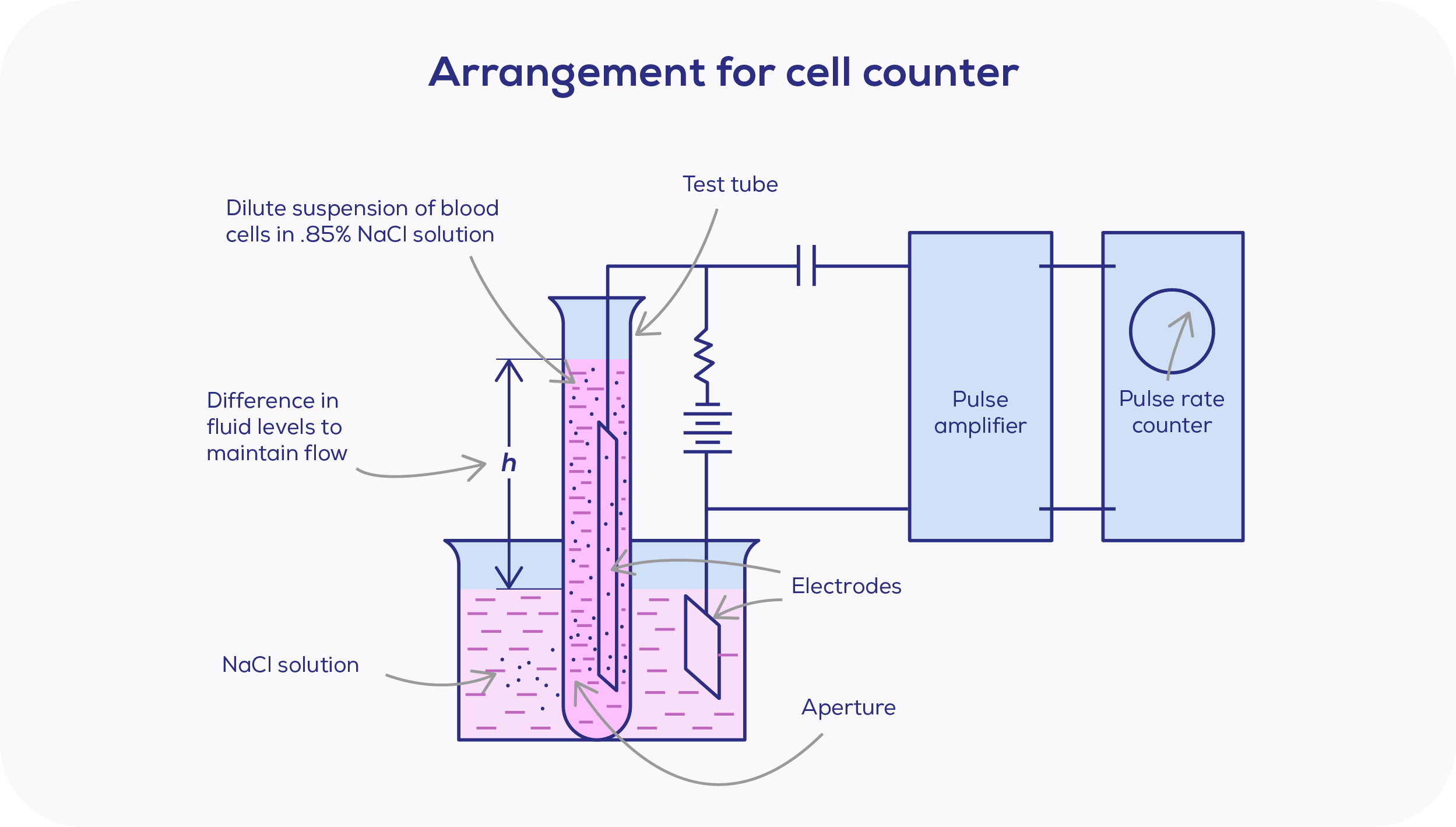
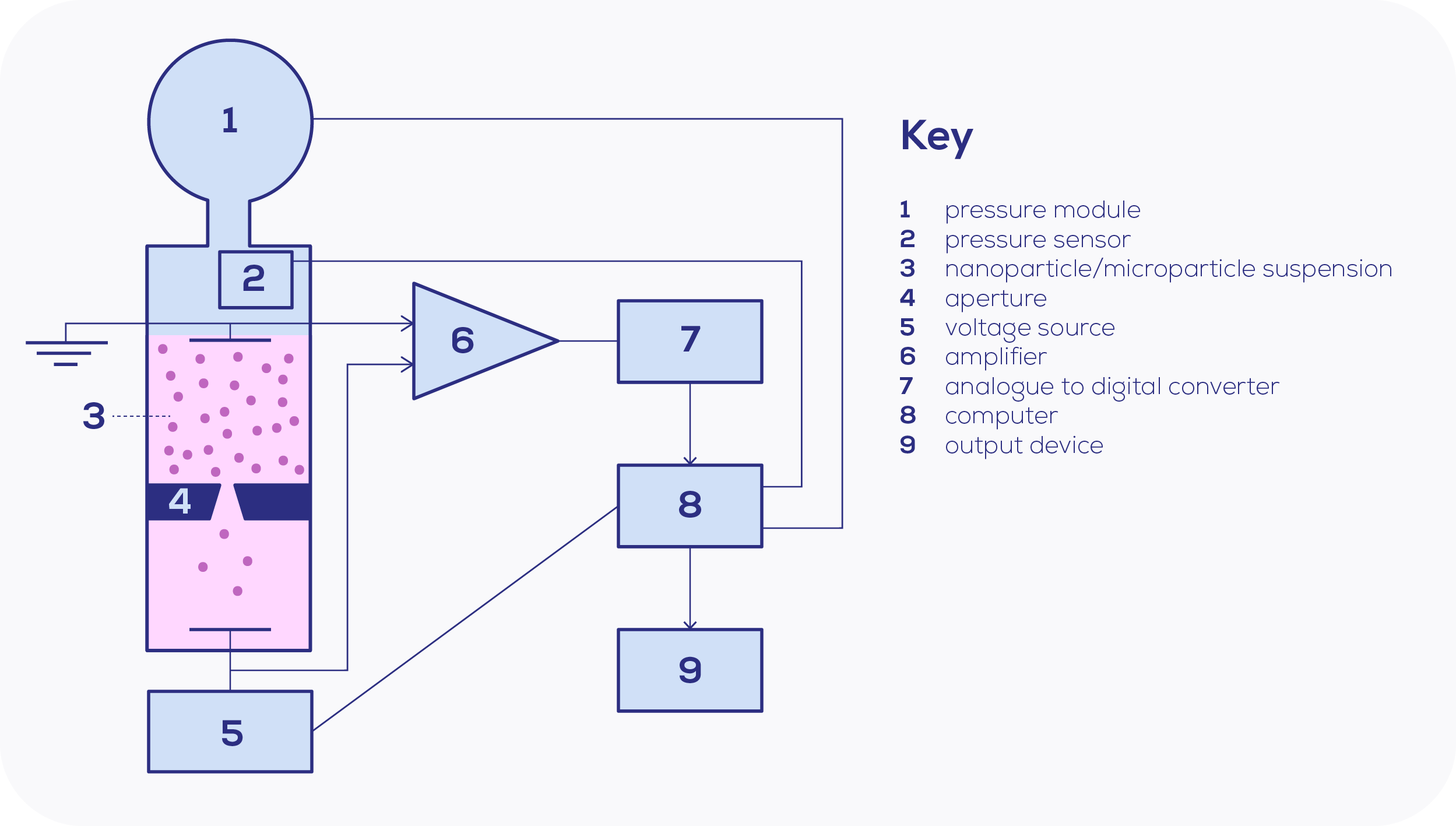
The principle applied – resistive pulse sensing of nanoparticles
The Coulter counter was utilised in many industries including the medical, pharmaceutical, and cosmetic fields. In the 70's, DeBlois & colleagues first reported applying the Coulter method to nanoparticles, which are defined as those between 1-100 nm in diameter.3,4 Below this range is the molecular realm, where nanoparticles are left behind. These days, we are becoming increasingly familiar with nanoparticles such as extracellular vesicles, lipoproteins and viruses. However, during the development of the Coulter method, nanoparticles were less well studied due to their small size and the lack of appropriate methods for characterisation.
The turn of the milennia introduced greater leaps to nanoparticle research, as nanopore fabrication improved. Essentially, nanopores are Coulter's aperture applied to the nanoscale, and Coulter's setup is strikingly similar to today's. The ions within the electrolyte solution carry the current through the nanopore, based on a voltage bias produced by Ag/AgCl electrodes. When a particle enters, this disrupts the current sensed at the nanopore, leading to a detected drop in current as the resistance is increased temporarily. This observed drop in current is known as a "blockade".
As this information is plotted on a chronometric graph, the blockade signal will be shown as a sharp spike, which can be used to determine particle characteristics:
- The magnitude of the spike is indicative of the particle diameter (i.e. size of resistance).
- The frequency of spikes is representative of particle concentration (number of particles entering the pore).
- The spike duration is representative of zeta potential, or surface charge density – as the time spent in the pore is reflective of the particle's charge/attraction to the electrode.
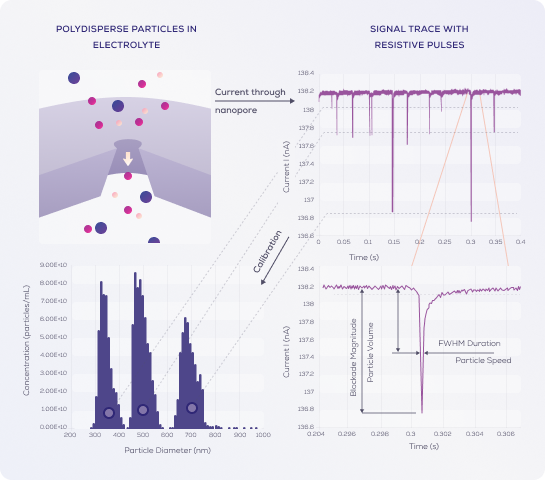
Blockades are recorded as nA, but need to be converted to nanometres to give the particle diameter. To accurately calculate particle diameter, concentration and charge, standardised calibration particles are used – with known size, concentration and zeta potential.
To determine sample particle size, the equation used is di=Kd ∛(Ai ); where di = size of sample particle, Kd = calibration constant and Ai = pulse height of the sample particle.
For particle concentration, the equation used to determine this is C = KC ((Δfp)/(ΔP)); where C = concentration, KC = calibration constant, fp = pulse frequency, and P = pressure.
Nanopores and their many considerations
When it comes to pore selection, geometry, material, and size are important considerations.5,6
Nanopore geometry
Nanopores can come in differing shapes, including cylindrical or conical. The nanopore shape can determine aspects such as the signal-to-noise ratio – which is crucial for accurate particle detection. Pore geometry can alter the number of event frequencies – that is, the number of nanoparticles that enter the pore. The event frequency is related to the various forces that affect particles, such as convection, electrophoresis, electroosmosis, dielectrophoresis, and drag. How these forces interact with nanoparticles depends on the shape of nanopore used.
Nanopore material
Nanopores can differ by materials also. There are three main types: solid-state, biological, and polymer. Choosing the appropriate material is important for a variety of reasons, including mitigating electrical noise that determines the nanopore's lower end detection limit.
Both pore geometry and material can have a profound effect on the pore's wetting protocol. Wetting of the nanopore is a very important step, and its process includes replacing trapped air within the nanopore with the electrolyte solution to bridge the upper and lower electrodes. It is a key step to ensure accurate and replicable TRPS measurements.
Nanopore size
The nanopore’s size itself must be carefully considered and is determined by the sample particle size to be characterised. While nanoparticles are defined as particles under the size of 100 nm, current limitations in nanopore fabrication means measurement of particles smaller than 40 nm in diameter are unable to be determined. This means that we are currently restricted to 40 nm and above for nanoparticle measurements.
Tunability of tunable resistive pulse sensing
With Tunable Resistive Pulse Sensing (TRPS), the nanopore diameter is adjustable through stretching in the horizontal plane, so that the pore size can be optimised for the sample's particles of interest.7
However, nanopore size is not the only tunable variable; tunability also comes in the form of adjustable voltage and pressure. The ability to manipulate the applied voltage and pressure allows for control over the forces which determine the frequency of a particle translocating through the pore. Being able to adjust these three variables allows for fine-tuning and careful optimisation for your sample. Importantly, TRPS allows accurate size and concentration measurements, and the option of measuring zeta potential – a feat most other techniques are not capable of.
Ready for high-resolution characterisation? Learn about the Exoid here