In recent years there has been an explosion of interest into plant extracellular vesicles (EVs) and, indeed, plant EV-like nanoparticles. Part of this interest has come from plant biologists who seek to understand the role that EVs naturally play in plants, especially in their response to pathogens. Despite their origins, plant EVs (and, of course, plant EV-like nanoparticles) are not solely the purview of plant biologists. If your research is of more of the mammalian kind, then there is perhaps just as much reason to study plant EVs, with both plant EVs themselves (and plant EV-like nanoparticles) showing therapeutic potential in their own right, as well showing potential as drug delivery vectors.
In this article, we will discuss the state of the plant EV field and how outdated isolation techniques can be improved upon to grow towards a brighter future. But first, why do we keep specifying ‘(and plant EV-like nanoparticles)’ every time we mention plant EVs?
A field full of controversy: divergent pre-processing
The reason for the parentheses boils down to a quiet dispute in the plant EV (and EV-like nanoparticle) literature. Both terms co-exist in a field fractured by pre-processing methodology. In this context, pre-processing refers to the process by which solid plant tissue is rendered into a liquid sample for EV isolation. You can’t exactly draw a blood sample from a begonia. This poses an interesting question. Does disrupting the tissue structure simply liberate existing EVs or does it damage cells to create EV-like particles in the process?
We looked through the currently published plant EV (and plant EV-like nanoparticle) literature to determine which methods of pre-processing were most common (Figure 1). Coming in overwhelmingly in the top spot is blending. Reading these papers, it is clear that the time spent blending varies wildly between studies, with the longest being over 10 minutes of blending3. However, logic would suggest that the amount of time spent blending may be inversely proportional to cell integrity. Whilst we have no direct comparison, a 2022 paper described the blending of watermelon fruit flesh for a single second, finding that this resulted in an EV isolate which was free from contaminating proteins and DNA from the nucleus, endoplasmic reticulum and mitochondria, suggesting minimal cell disruption.1 As such, a minimal blending time could allow the tissue structure of fruits to be disrupted enough to isolate EVs, without bursting cells open.
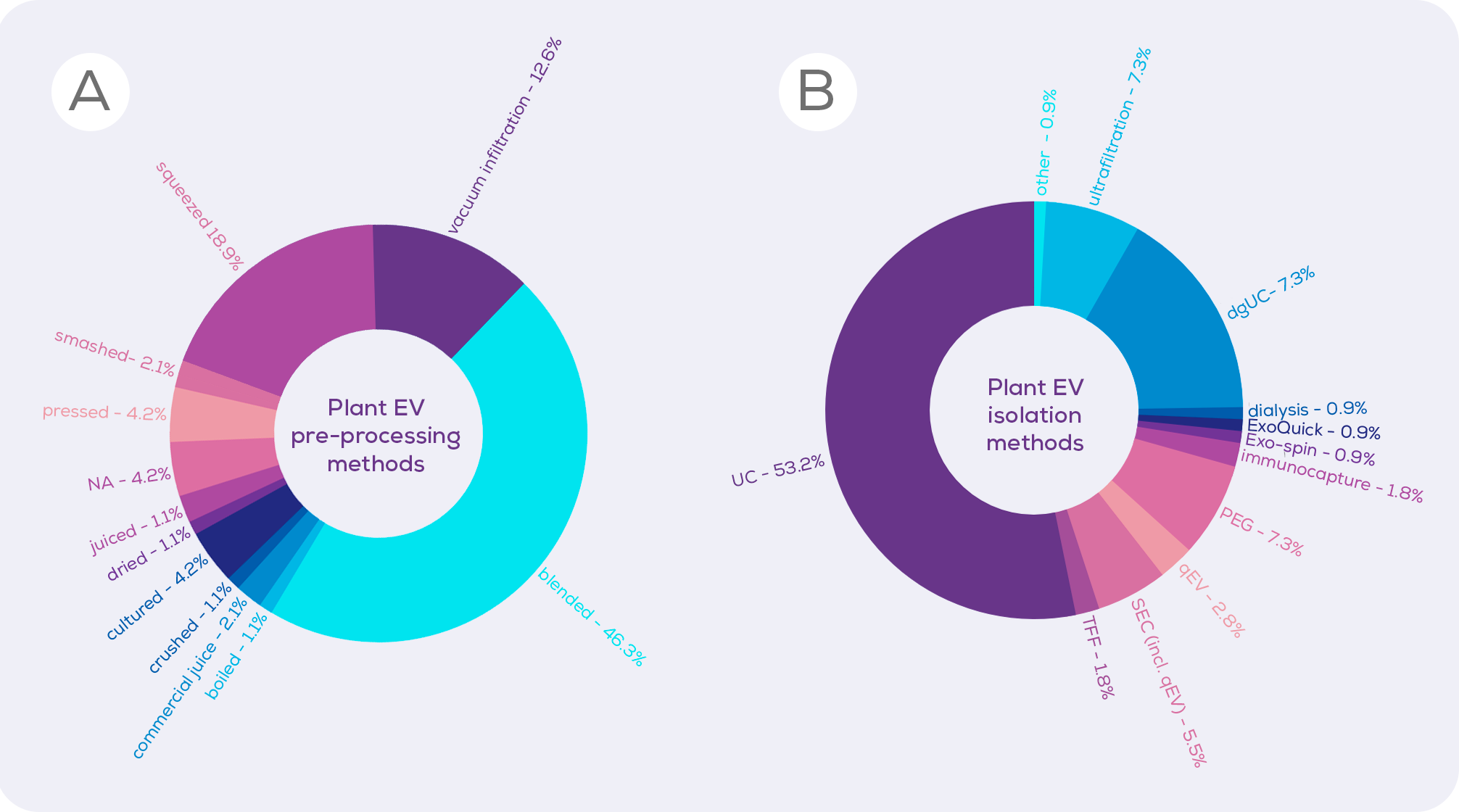
Perhaps rightly in some circumstances and unfairly in others, some researchers dismiss studies using blending or the even squeezing of citrus fruits, assuming that these methods create artificial EVs.3,4 Instead, they – and they are primarily the plant biologists – favour vacuum infiltration, which involves forcing liquid into plant tissue under pressure in order to extract the apoplast (essentially, the apoplast is the plant version of interstitial fluid, existing between and within the cell walls of plant cells). Vacuum infiltration is billed by those who favour it as a gentle technique which does not create artificial EVs. And, cell disruption is likely to be lower than would occur from 10 minutes of blending.
However, there is a drawback of vacuum infiltration which should be acknowledged; vacuum infiltration may cause cell permeation. With plasmid entry into cells via non-endocytic or phagocytic means, there is the potential for membrane damage, the creation of artificial EVs, and leakage of cellular organelle material.5,6 As such, neither technique is perfect.
But what if it didn’t matter if artificial EVs were created alongside the bona fide ones? For nutrition research, disruption to the plant structure is essential to mimic what happens in food preparation and mastication. And, from a therapeutic perspective, it can be argued that the origin of the vesicles doesn’t matter if the isolated particles have the desired effect. Only when it comes to pure plant biology is the issue of ‘artificially generated EVs’ a more important consideration. Any task force created for the improvement and promotion of plant EV research should therefore include a variety of researchers, not just one subgroup. Only then can methodology be improved all around.
A field in bloom: the fast-growing field of plant EV biology
Now that we’ve gotten that controversy out of the way, lets settle into the science and discuss some of the discoveries made about plant EVs (yes, we’re just calling them all that for now) so far.
EVs were first isolated from the apoplastic fluid of plants in 2009.7 At least some of these EVs are likely derived from the fusion of multivesicular bodies with the plant plasma membrane, making them exosomes.8 So far, so mammalian-like. They even contain tetraspanins, the poster-children of EV proteins.11-13 However, this is where things begin to diverge. The lipids of plant EVs are distinct from those of mammalian EVs, with high levels of certain phospholipids and ceramides potentially influencing internalisation and function.9,10 In addition, plant EVs contain plant specific proteins. In addition to the TET8 and TET9 tetraspanins, the syntaxin PEN1 is also common in plant EVs, theorised to derive from an exosome-like pathway.11-13 Other pathways likely also exist in plants, with EVs possibly also deriving from the excyst-positive organelle14 and from plastids1,3, meaning that they contain proteins from these plant-specific organelles. Alongside proteins, plant EVs also contain plant-specific small molecules15 and both small and tiny RNAs16,17. These RNAs and other plant EV components have anti-fungal properties, suggesting a role in response to pathogens.13,17,18 In addition, plant EVs (and EV-like nanoparticles) contain antioxidants.2,19,20
Despite this knowledge of plant EV markers and characterisation, functional information on plant EVs in plants is somewhat limited. However, the mammalian EV world has taught us that truly specific markers for EV subtypes are likely non-existent. This is due to the promiscuity of proteins, which don’t quite fall into the neat, delineated jobs and subcellular locations that we would like them to. A more reliable – or at the very least, complementary – method of delineating plant EV populations would be to avoid markers entirely, instead turning to single vesicle analysis methods which are based solely on measuring the physical properties of nanoparticles. Tunable Resistive Pulse Sensing (TRPS) with the Exoid allows you to simultaneously visualise the size and charge of individual vesicles and, therefore, different vesicle populations within a mixture, all in a label-free manner.
Flora and fauna: plant EVs as mammalian therapeutics
Alongside fundamental characterisation work, there is a portion of the field focused on the functional roles and therapeutic potential of plant EVs. While therapeutic interest in plant EVs began with the identification of antioxidant properties, it has quickly diversified and a number of studies have indicated the therapeutic value of plant EVs.2,19,20 For instance, taken orally, plant EVs were found to protect against experimentally-induced colitis in mice.15,21-23 Dietary plant EVs also alter intestinal epithelial cell basal secretions (i.e. secretions towards the direction of the blood, not the intestinal lumen), altering the function of distal organs such as the placenta and liver.1,24 In vitro and in vivo, plant EVs have also shown anticancer25-30 and antiaging31,32 properties, suggesting that the therapeutic potential of plant EVs is substantial.
In addition to their natural medicinal properties, plant EVs are also being trialled as drug delivery vectors. This stems from their relative low production cost, good tolerability and the absence of zoonotic or human viruses which may be present in mammalian EVs.33,34 However, without large scale clinical trials, we cannot be sure that immunogenicity won’t be a problem for plant EVs. Such clinical trials involving drug loaded plant EVs have already begun (e.g. Trial NCT04879810), and many more in vitro and in vivo preclinical studies have been reported.35
Regardless of whether your plant EV therapeutic is in a “wild-type” or drug-loaded state, isolating plant EVs in a pure and scalable fashion is essential for taking a treatment from culture flask to a patient.
Sowing the seeds of improved isolation methodology with size exclusion chromatography
Ultracentrifugation (UC) is, unfortunately, the current method of choice for isolating plant EVs (Figure 1) and precipitation (e.g., polyethylene glycol; PEG) is also popular. Compared to sized exclusion chromatography, however, these techniques are inferior in terms of EV purity and integrity, isolating large amounts of contaminating proteins, and even damaging EVs. When UC, PEG and size exclusion chromatography-based qEV columns were compared for the isolation of EVs from cabbages, qEV columns were clearly superior in terms of purity (i.e. vesicles/µg protein) without compromising vesicle yield.33
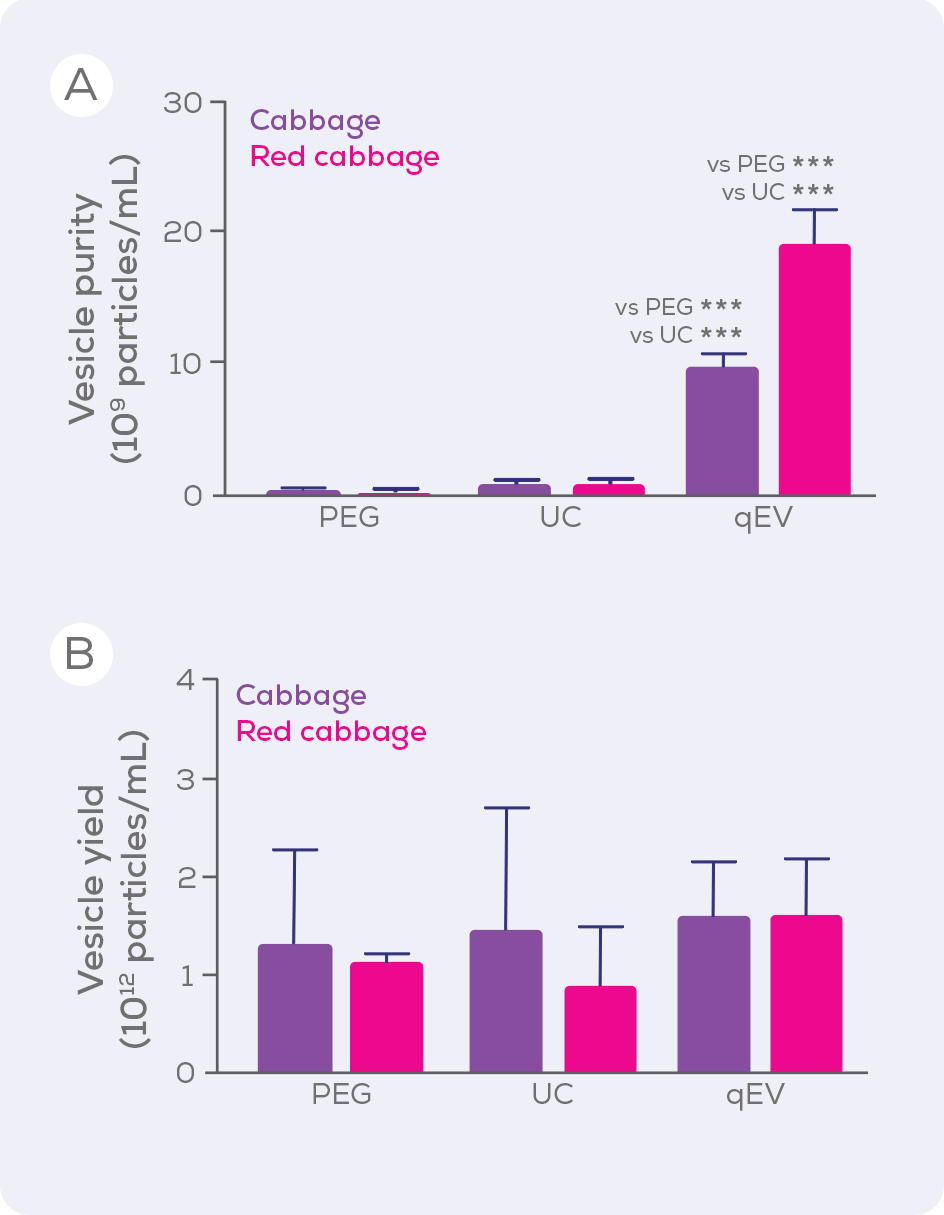
Aside from purity and yield, another benefit of qEV columns is their versatility. Gen 2 qEV columns are made using a high-performance resin specifically designed for EV isolation and are available in a variety of sizes to suit a wide range of sample loading volumes – making them compatible with your plant EV research, whether you are isolating from a single seed or a watermelon. The larger qEV2, qEV10 and qEV100 are respectively optimised for samples sizes of 2, 10 and 100 mL and so are particularly suited to large scale EV isolation. Beyond these standard columns, we offer customised EV separation solutions to pair our technology with your workflow and allow you to scale reproducible, pure EV isolations to an industrial scale.
Get in touch today to find out how we can help you achieve your plant EV goals!